Research
My research in nuclear astrophysics focuses on constraining the properties of the neutron star interior. In particular, our research group uses neutron star observations, nuclear mass models, and reaction networks to investigate the neutron star crust.
Late time cooling of neutron star transients (2016)
An accretion outburst onto a neutron star transient heats the neutron star's crust out of thermal equilibrium with the core. After the outburst the crust thermally relaxes toward equilibrium with the neutron star core and the surface thermal emission powers the quiescent X-ray light curve. Crust cooling models predict that thermal equilibrium of the crust will be established approximately 1000 days into quiescence. Recent observations of the cooling neutron star transient MXB 1659-29, however, suggest that the crust did not reach thermal equilibrium with the core on the predicted timescale and continued to cool after approximately 2500 days into quiescence. Because the quiescent light curve reveals successively deeper layers of the crust, the observed late time cooling of MXB 1659-29 depends on the thermal transport in the inner crust. In particular, the observed late time cooling is consistent with a low thermal conductivity layer near the depth predicted for nuclear pasta that maintains a temperature gradient between the neutron star's inner crust and core for thousands of days into quiescence. As a result, the temperature near the crust-core boundary remains above the critical temperature for neutron superfluidity and a layer of normal neutrons forms in the inner crust. We find that the late time cooling of MXB 1659-29 is consistent with heat release from a normal neutron layer near the crust-core boundary with a long thermal time.
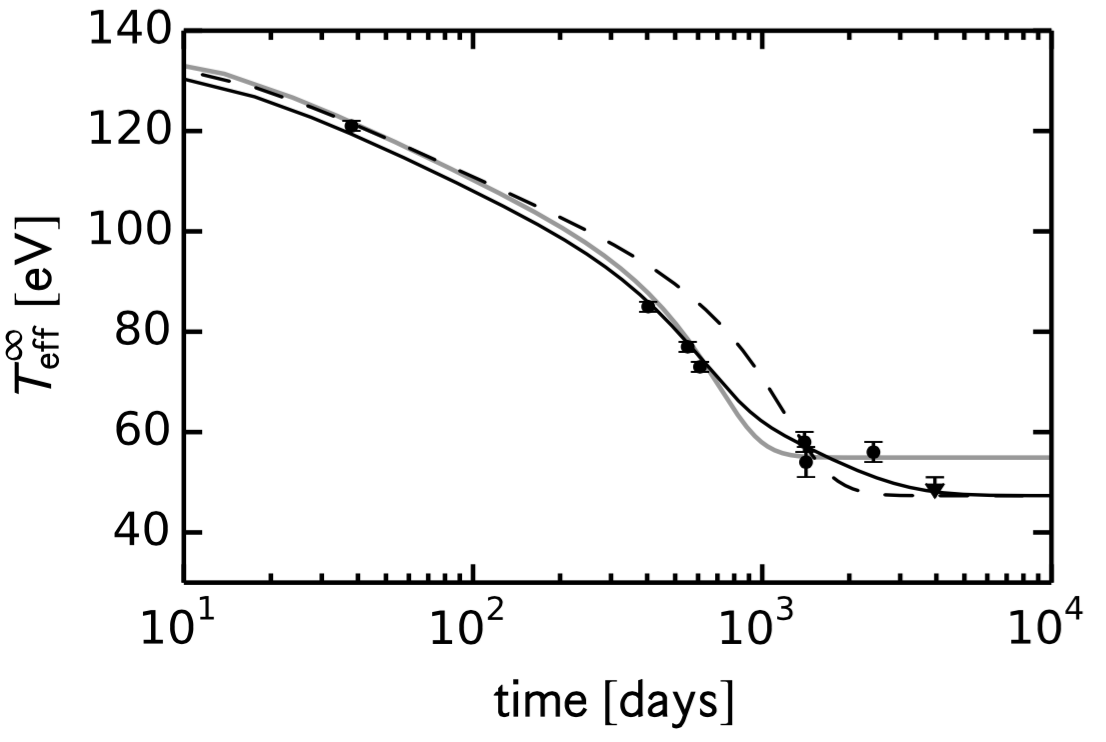
Cooling models for MXB~1659-29. The solid gray curve is a model that uses Q_imp=2.5 throughout the entire crust and T_core = 4E7 K. The solid black curve is a model with Q_imp=20 for rho>8E13 g/cc, Q_imp=1 for rho< 8E13 g\cc, T_core = 3E7 K, and using the G08 pairing gap. The dashed black curve uses the same Q_imp as the solid black curve, but with the S03 pairing gap.
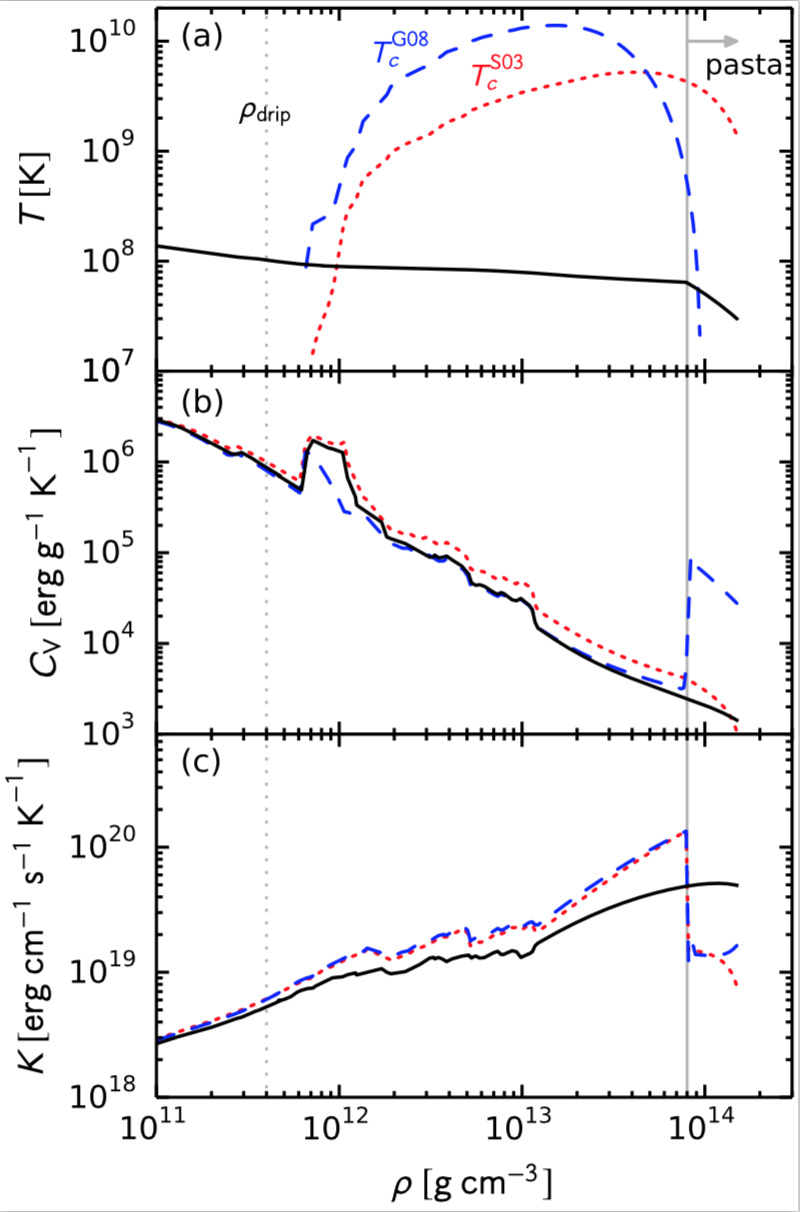
Cooling models for MXB~1659-29. The solid gray curve is a model that uses Q_imp=2.5 throughout the entire crust and T_core = 4E7 K. The solid black curve is a model with Q_imp=20 for rho>8E13 g/cc, Q_imp=1 for rho< 8E13 g\cc, T_core = 3E7 K, and using the G08 pairing gap. The dashed black curve uses the same Q_imp as the solid black curve, but with the S03 pairing gap.
Investigators
A. Deibel, A. Cumming, E. F. Brown, and Sanjay Reddy
Publications
Support
Support for A. D. and E. F. B. was provided by the National Aeronautics and Space Administration through Chandra Award Number TM5-16003X issued by the Chandra X-ray Observatory Center, which is operated by the Smithsonian Astrophysical Observatory for and on behalf of the National Aeronautics and Space Administration under contract NAS8-03060. A. D. and E. F. B. are also supported by the National Science Foundation under Grant No. AST-1516969. A. D. is also grateful for the support of the Michigan State University College of Natural Science Dissertation Completion Fellowship. A.C. is supported by an NSERC Discovery grant, is a member of the Centre de Recherche en Astrophysique du Quebec (CRAQ), and an Associate of the CIFAR Cosmology and Gravity program. S. R. was supported by the U.S. Department of Energy under Contract No. DE-FG0200ER41132. This material is based upon work supported by the National Science Foundation under Grant No. PHY-1430152 (JINA Center for the Evolution of the Elements).
Thermal relaxation of neutron star crusts (2015)
During an accretion outburst, a neutron star in a low-mass X-ray binary will have its crust heated out of thermal equilibrium. This occurs as accreted material compresses the existing crust, which then releases heat through electron captures and pycnonuclear fusion reactions. When accretion halts, the neutron star crust cools over the course of thousands of days. We investigate the crust cooling using a thermal evolution simulation of the crust that takes into account the crust microphysics, such as the composition and thermal conductivity. Model fits to the quiescent lightcurve of MAXI J0556-332 suggest that there is more heat deposited during the accretion outburst than would be expected from compressional heating sources. In this study, we put limits on the amount of extra heating needed to reconcile observations and models. Investigations into the source of this extra heating are ongoing.
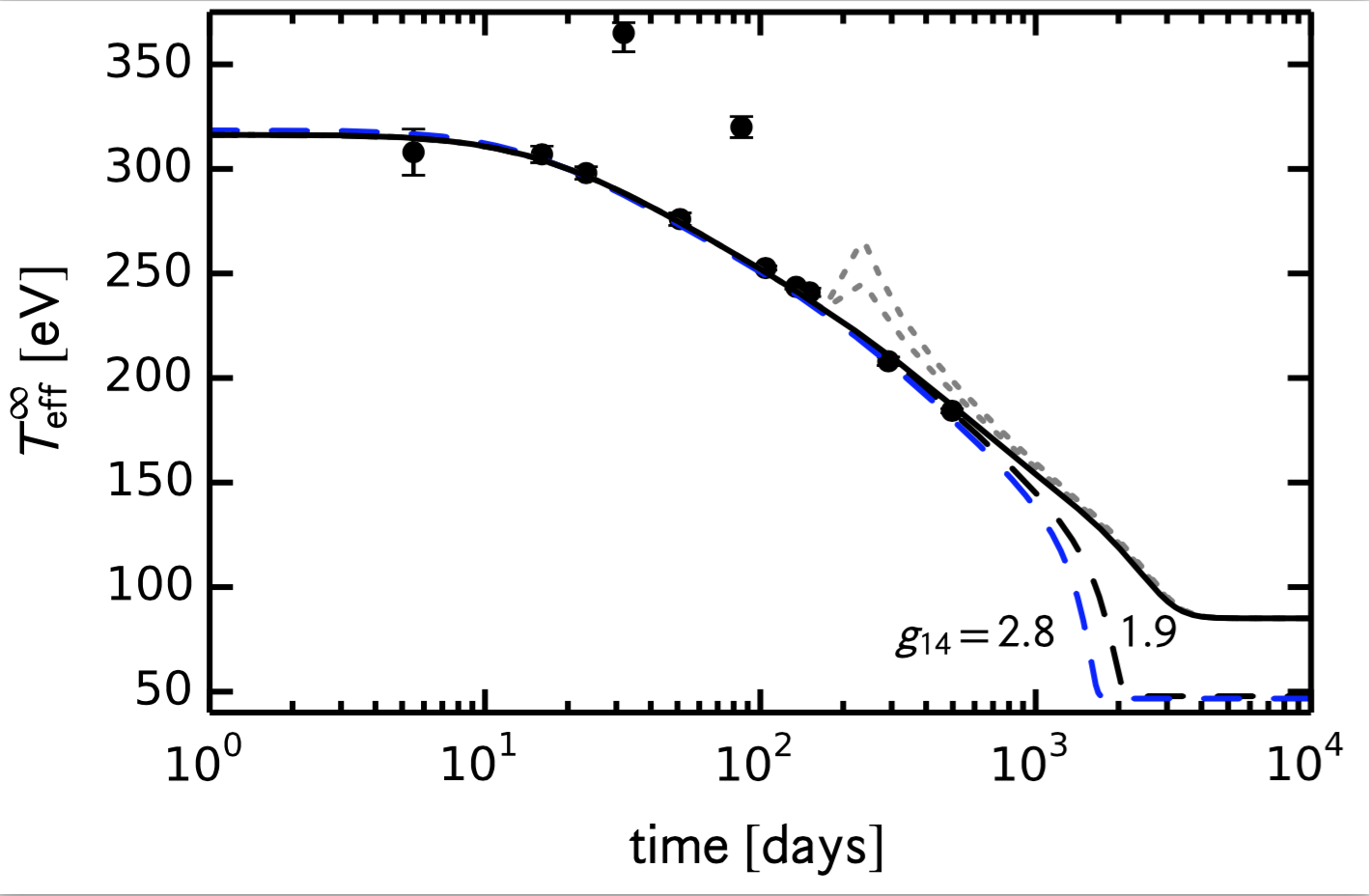
Thermal relaxation models of the quiescent behavior of MAXI J0556-332. X-ray observations (black points) are from Homan et al. 2014. The black curves are for a model with M=1.4 M⊙ and R = 12 km, with Tc=1E8 K (solid curve) and Tc=3E7 K (dashed curve). The color curves are for a M=1.4 M⊙ and R=10 km model (blue), and a M=1.2 M⊙ and R=13 km model (red). The black dotted curve is for the reheating event, which occurred 170 days into quiescence and lasted about 60 days.
Investigators
A. Deibel, A. Cumming, E. F. Brown, and D. Page
Publications
Support
Support for A.D. and E.F.B. was provided by the National Aeronautics and Space Administration through Chandra Award Number TM5-16003X issued by the Chandra X-ray Observatory Center, which is operated by the Smithsonian Astrophysical Observatory for and on behalf of the National Aeronautics and Space Administration under contract NAS8-03060. A.C. is supported by an NSERC Discovery grant, is a member of the Centre de Recherche en Astrophysique du Quebec (CRAQ), and an Associate of the CIFAR Cosmology and Gravity program. D. P. is partially supported by a grant from Mexican Conacyt (CB-2009-01, No. 132400). The authors are grateful for support received as part of the International Team on Neutron Star Crusts by the International Space Science Institute in Bern, Switzerland. This material is based upon work supported by the National Science Foundation under Grant No. PHY-1430152 (JINA Center for the Evolution of the Elements). The authors thank Zach Meisel and Hendrik Schatz for useful discussions.
Urca shell cooling in neutron stars (2014)
The crust of an accreting neutron star is heated by nuclear reactions as accreted material is compressed deeper into the crust. We find that strong neutrino cooling occurs in the outer crust as pairs of neutron-rich nuclei undergo electron capture and β-decay cycles. These Urca pairs thermally decouple the surface and the deeper crust. In the absence of a heat flux into the surface layers an unknown local heating mechanism is required to ignite superbursts.
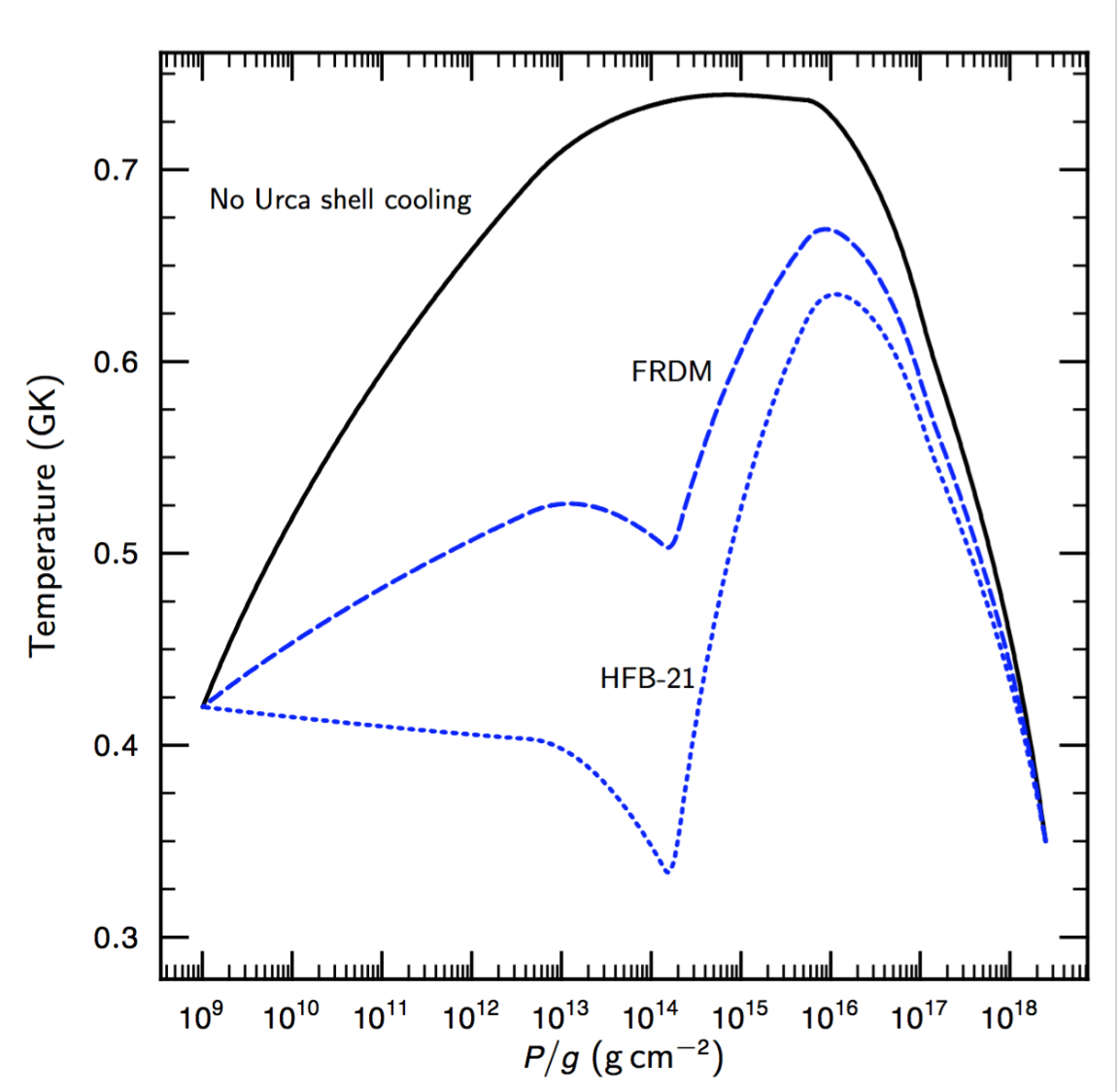
Temperature as a function of depth in the accreted neutron star crust for different Urca shell cooling strengths. Here, y~P/g is a proxy for depth, where P is the pressure, g the local gravitational acceleration. In the absence of Urca shell cooling, the peak local temperature reaches 0.73 GK (solid curve). With the addition of cooling using the HFB-21 mass model and a superburst ash composition (blue dotted line), a local temperature minimum, T = 0.33 GK, appears at the location of the Urca shell. Indeed, for these conditions the temperature at the Urca shell is lower than that at the upper boundary, so that a temperature inversion develops. Even for the much lower Urca shell emissivity of the FRDM mass model (blue dashed line), the temperature at the depth of the superburst ignition is <5E8 K, which is inconsistent with typical superburst ignition conditions. Nature 505, 62 (2014).
Investigators
H. Schatz, S. Gupta, P. Möller, M. Beard, E. F. Brown, A. T. Deibel, L.R. Gasques, W. R. Hix, L. Keek, R. Lau, A. W. Steiner, & M. Wiescher
Publications
Support
This project was funded by NSF grants PHY 08-22648 (Joint Institute for Nuclear Astrophysics) and PHY 06-06007. A.S. is supported by INT DOE grant DE-FG02-00ER41132. EFB is supported by NSF grant AST 11-09176. PM is supported by the National Nuclear Security Administration of the U. S. Department of Energy at Los Alamos National Laboratory under Contract No. DE-AC52-06NA25396. We thank D.M. Yakovlev, P. Shternin, and S. Reddy for discussions and comments on the manuscript.
Magnetar giant flares (2013)
Isolated neutron stars with strong magnetic fields, or magnetars, provide a unique opportunity to learn about the masses and radii of neutron stars. Giant flares from these objects are thought to occur following a starquake on the magnetar surface. Quasi-periodic oscillations are observed in the emission of these giant flares and could be associated with an oscillating crust. To learn about masses and radii of magnetars we perturb a crust model to reproduce the observed oscillation frequencies. Matching observations using a model with the surface dipole field of SGR 1806-20 (B=2.4E15 G) we obtain the approximate values of M = 1.25 M⊙ and R = 12.4 km.
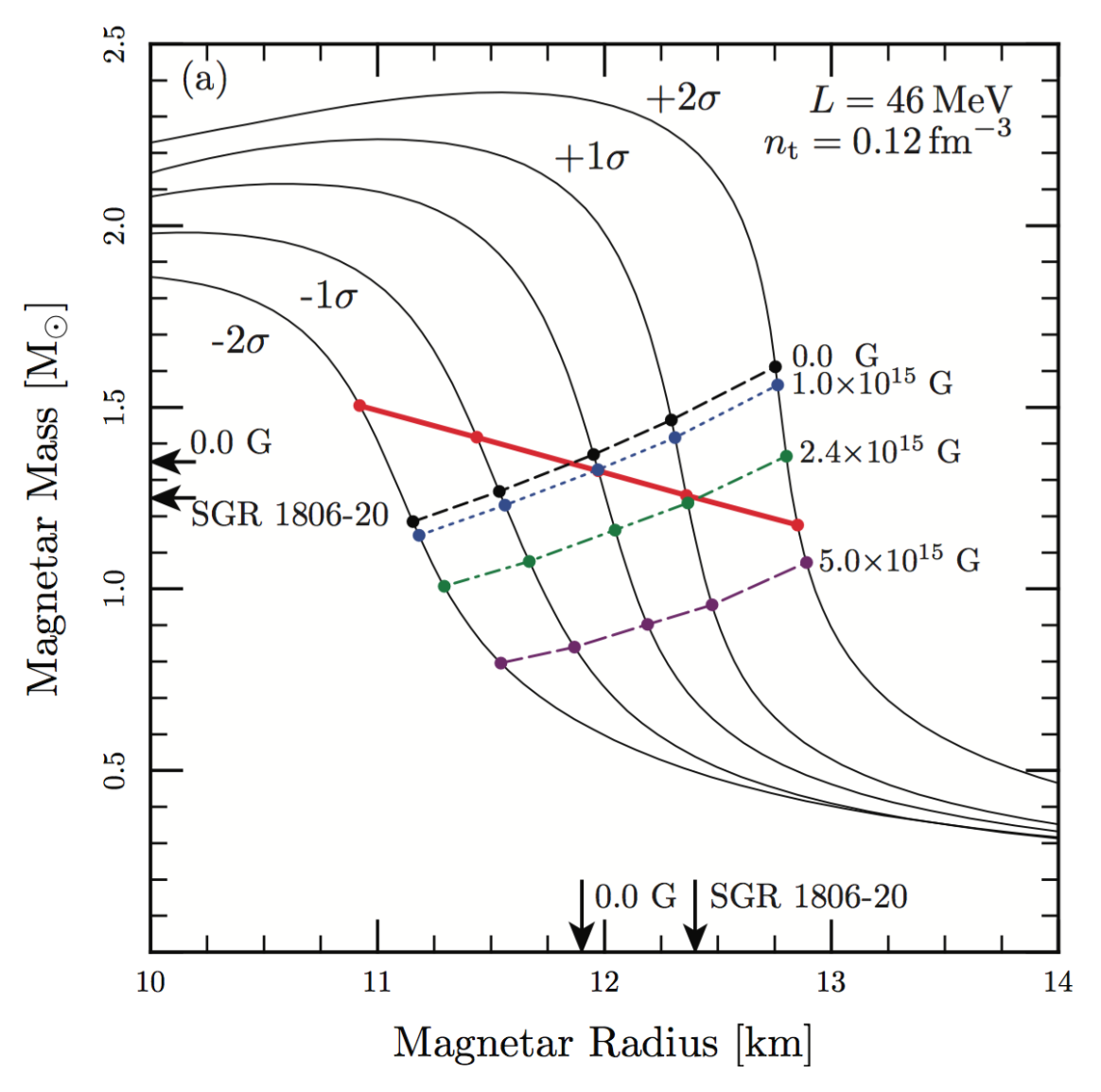
Magnetar mass as a function of radius for the core EOS probability distribution from Steiner et al. 2012. Frequencies are evaluated using the SLy4 crust EOS for two different crust-core transition densities, n = 0.12 fm−3. The thick red solid line indicates masses and radii for which the fundamental mode has a frequency of 29 Hz. The black short-dashed line indicates masses and radii for a 626 Hz harmonic mode and B = 0 G. Masses and radii from 626 Hz harmonic modes with magnetized crusts are labeled accordingly. Arrows indicate masses and radii that match both the fundamental and the harmonic modes for the field-free case and the case with the magnetic field of SGR 1806-20 (B = 2.4E15 G).
Investigators
Alex T. Deibel, Andrew W. Steiner, and Edward F. Brown
Publications
Support
This work is supported by NASA ATFP grant NNX08AG76G, U.S. DOE grant DEFG02-00ER41132, Chandra grant TM1-12003X, NSF grant 1109176, and by the Joint Institute for Nuclear Astrophysics at MSU under NSF PHY grant 08-22648. The authors thank Andrew Cumming and Sanjay Reddy for useful discussions.